Summary: Researchers unveiled the most extensive single-neuron projectome database to date, featuring over 10,000 mouse hippocampal neurons.
The study provides an unprecedented view of the spatial connectivity patterns at the mesoscopic level, crucial for understanding learning, memory, and emotional processing in the hippocampus. By employing machine learning algorithms for categorizing axonal trajectories and integrating spatial transcriptome data, researchers identified 43 distinct projectome cell types, revealing intricate projection patterns and soma locations’ correspondence to projection targets.
This work, accessible via the Digital Brain CEBSIT portal, lays the structural foundation for advancing our knowledge of hippocampal functions and their molecular underpinnings.
Key Facts:
- The study reconstructed whole-brain axonal morphology of over 10,000 mouse hippocampal neurons, creating the world’s most extensive single-neuron projectome database.
- Researchers used machine learning to analyze morphological similarities among neurons, identifying 43 distinct projectome cell types.
- The integration of projectome cell types with spatial transcriptome data revealed potential molecular and circuit targets for hippocampal functions, all accessible through a dedicated online platform.
Source: Chinese Academy of Science
A study published in Science on Feb. 1 reported a comprehensive database of single-neuron projectomes consisting of over 10,000 mouse hippocampal neurons, thus revealing the spatial connectivity patterns of mouse hippocampal neurons at the mesoscopic level.
The study was conducted by teams from the Center for Excellence in Brain Science and Intelligence Technology (CEBSIT), the Institute of Neuroscience of the Chinese Academy of Sciences (CAS), the HUST-Suzhou Institute for Brainsmatics, Hainan University, the Kunming Institute of Zoology of CAS, Lingang Laboratory, and the Shanghai Center for Brain Science and Brain-Inspired Technology.
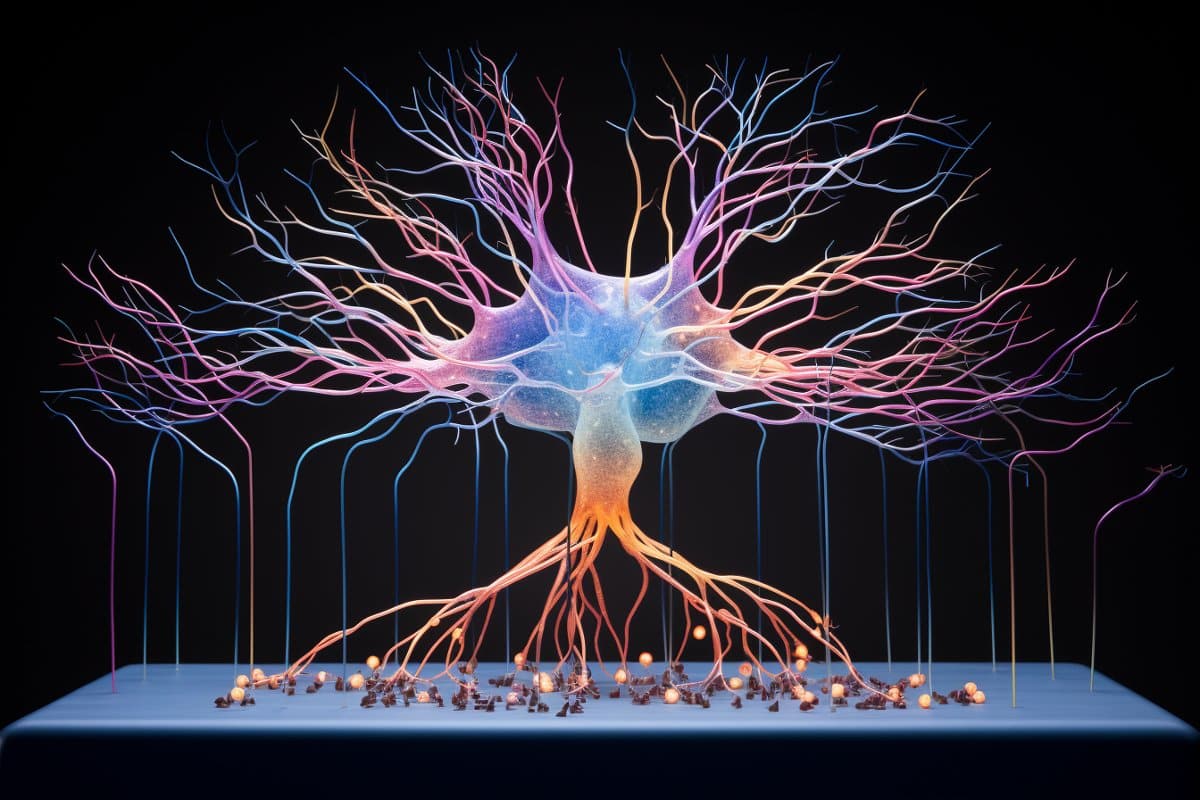
The hippocampus serves as an essential brain region for learning and memory as well as various brain functions such as spatial cognition and emotional processing. It is one of the most extensively studied brain regions.
Hippocampal neurons project widely to the brain-wide targets; thus, it is critical to investigate the projection patterns of hippocampal neurons at the single-neuron level.
This study reconstructed the whole-brain axonal morphology of over 10,000 neurons in the mouse hippocampus at a single-cell resolution with the neuronal cell bodies covering all subregions and multiple locations along different hippocampal axes, making this the most extensive single-neuron projectome database in the world.
This study took an innovative approach to categorize axonal trajectories with machine learning algorithms, thus allowing for a more efficient analysis of the morphological similarities among 341 projection patterns for mouse hippocampal neurons and ultimately identifying 43 distinct projectome cell types. It also incorporated the spatial transcriptome of mouse CA1 areas.
Based on these analyses, the study was able to elucidate the axonal projection pathways of hippocampal neurons along the anterior-posterior axis and reveal new projection patterns of hippocampal neurons. It also outlined the correspondence between hippocampal neuron soma locations and projection targets, and revealed basic organization principles of bilateral projections.
Furthermore, correlation analysis of projectome cell types and spatial transcriptome data identified spatial correspondence between various genes and projectome subtypes, providing potential molecular and circuit targets for hippocampal functions.
Taken together, this study provides a structural basis for future studies of hippocampal functions and deciphers the potential correspondences between their soma locations, gene expression, and circuitry functions.
The database for the hippocampal single-neuron projectomes, along with the database on the hippocampal longitudinal axis and spatial transcriptomes, are now publicly accessible through the Digital Brain CEBSIT portal (https://mouse.digital-brain.cn/hipp).
To facilitate broader usage of the databases, a team from the Computing and Data Center of CEBSIT has developed a website to integrate data visualization, user interface, online analysis, and data downloads.
Abstract
Whole-brain spatial organization of hippocampal single-neuron projectomes
INTRODUCTION
In the brain circuitry, a single neuron could broadcast output signals to other neurons located in nearby or distant areas. Therefore, understanding the spatial organization of axon projections at the single-cell level is crucial for elucidating the neural circuitry underlying various brain functions. As a brain structure essential for learning, memory, cognition, stress responses and emotional behaviors, it is known that the hippocampus (HIP) is widely connected with various brain areas, including the cortex, thalamus, hypothalamus, olfactory areas, and amygdala. However, it remains unclear how single HIP neurons project to brain-wide target areas and how a single-neuron projectome can be specified by the soma location within the HIP.
RATIONALE
To reconstruct single-neuron projectomes of the mouse HIP, we combined sparse-labeling methods with fluorescence micro-optical sectioning tomography and reconstructed the soma, axon arbors, and dendrites of individual neurons. We used a wide range of analytic tools for the projectome analysis and created a state-of-art web interface to visualize the projectome data.
RESULTS
We have created an open and comprehensive database with 10,100 single-neuron projectomes throughout the HIP, which allows interactive query, visualization, and analysis of reconstructed brain-wide projectomes. We classified HIP neurons into 341 projection patterns, and then 43 projectome subtypes, based on their axon morphology and brain-wide target areas.
Our study revealed previously unknown axon projection patterns, target-dependent soma distribution within HIP subdomains, a general rule for bihemispheric projections, axon orientation rules for mossy fibers and Schaffer collaterals, and topographic correlation between axon arbors and soma location along HIP axes.
CONCLUSION
Single-neuron projectome analyses have provided unprecedented information on axon projection patterns at the single-cell resolution and elucidated the organizational principles of whole-brain connectivity of HIP neurons. Such knowledge could be further combined with gene expression data to define HIP neuron subtypes and serve as the structural basis for understanding their diverse and coordinated functions.